By eliminating the need for hardwiring to the electrical grid, battery-powered remote wireless devices are providing inexpensive and simple-to-install solutions that take enhanced data intelligence virtually everywhere.
Remote battery-powered devices enable real-time data and remote connectivity to maximize operational efficiency, improve quality control and product reliability, optimize supply chains and track assets. The most common applications include supervisory control and data acquisition (SCADA), process control, industrial robotics, memory back-up, asset tracking, safety systems, environmental monitoring, machine-to-machine (M2M) communication, machine learning and wireless mesh networks, to name a few.
The ideal battery
The heart of any wireless device is its battery. The importance of specifying the ideal battery grows exponentially with long-term deployments in remote locations and extreme environments, especially where battery replacement is either prohibitively expensive or impossible. Choosing the right battery is often the key to minimizing your total cost of ownership by reducing or eliminating the need for battery replacement throughout the lifetime of the device.
There are no one-size-fits-all solutions, as application-specific requirements dictate the ideal choice of battery. For example, if a wireless device is being deployed indoors at moderate temperatures, and where the battery is easily accessible for replacement, it could call for the use of an inexpensive consumer-grade alkaline or lithium battery. However, for hard-to-access locations, extreme environments, or in situations where a large-scale unplanned battery failure could be disastrous, specifying an ultra-long-life battery can be critical (Figure 1).Ultra-long-life batteries are generally more expensive than consumer cells, but the long-term savings will typically far outweigh the added cost if it lowers long-term maintenance expenses while reducing the risk of compromised data integrity and/or connectivity. Trade-offs are inevitable.
Specialized requirements
The typical low-power wireless device operates predominantly in a standby state, drawing micro-Amps of base current, alternating with high pulses in the multi-Amp range to power two-way wireless communications and other advanced functionality. To further conserve energy, these low-power devices also utilize a low-power communications protocol, such as WirelessHART, ZigBee or long-range (LoRa), along with low-power chip sets, as well as proprietary techniques designed to minimize energy losses during active mode.
There are also certain niche applications that require higher amounts of base current measurable in milliAmps, drawing enough average energy to prematurely exhaust a primary cell. As a result, these devices may require the use of an energy-harvesting device in combination with an industrial-grade rechargeable Lithium-ion (Li-ion) battery to store the harvested energy. Industrial-grade Li-ion batteries are available that can operate for up to 20 years and 5,000 full recharge cycles, whereas the typical consumer grade Li-ion cell may last roughly five years and 500 recharge cycles.
Various primary, non-rechargeable battery chemistries can be considered (Table 1), each offering unique benefits and disadvantages. At one end of the spectrum are inexpensive consumer-grade alkaline cells. These commonly available batteries deliver high-rate continuous energy but suffer from a variety of shortcomings, including an extremely high annual self-discharge rate of up to 60% per year, along with low capacity, low energy density, and low voltage. In addition, alkaline cells cannot withstand extreme temperatures due to a water-based chemistry.
Primary Cell | LiSOCL2 Bobbin-type with Hybrid Layer Capacitor | LiSOCL2 Bobbin-type | Li Metal Oxide Modified for high capacity | Li Metal Oxide Modified for high power | Alkaline | LiFeS2 Lithium Iron Disulfate (AA-size) | LiMnO2 Lithium Manganese Oxide |
Energy Density (Wh/Kg) | 700 | 730 | 370 | 185 | 90 | 335 | 330 |
Power | Very High | Low | Very High | Very High | Low | High | Moderate |
Voltage | 3.6 to 3.9 V | 3.6 V | 4.1 V | 4.1 V | 1.5 V | 1.5 V | 3.0 |
Pulse Amplitude | Excellent | Small | High | Very High | Low | Moderate | Moderate |
Passivation | None | High | Very Low | None | N/A | Fair | Moderate |
Performance at Elevated Temp. | Excellent | Fair | Excellent | Excellent | Low | Moderate | Fair |
Performance at Low Temp. | Excellent | Fair | Moderate | Excellent | Low | Moderate | Poor |
Operating Life | Excellent | Excellent | Excellent | Excellent | Moderate | Moderate | Fair |
Self-Discharge Rate | Very Low | Very Low | Very Low | Very Low | Very High | Moderate | High |
Operating Temp. | -55°C to 85°C, can be extended to 105°C for a short time | -80°C to 125°C | -45°C to 85°C | -45°C to 85°C | 0°C to 60°C | -20°C to 60°C | 0°C to 60°C |
Table 1: Various primary, non-rechargeable battery chemistries each offer unique benefits and disadvantages.
At the opposite end of the spectrum are more robust lithium chemistries. As the lightest non-gaseous metal, lithium features an intrinsic negative potential that exceeds all others, delivering the highest specific energy (energy per unit weight), highest energy density (energy per unit volume), and higher voltage (open-circuit voltage, OCV) ranging from 2.7 to 3.6 V. Lithium battery chemistries are also non-aqueous and thus less likely to freeze than alkaline cells (Figure 2).Within the lithium family, bobbin-type lithium thionyl chloride (LiSOCl2) batteries stand apart as being overwhelmingly preferred for ultra-long-life applications. This is due to a combination of unique factors, as bobbin-type LiSOCl2 cells deliver the highest capacity and energy density, which enables the use of fewer or smaller cells, an extended temperature range to survive extreme environments (-80 °C to +125 °C), and, most importantly, an incredibly low annual self-discharge rate, as low as 0.7% per year, which enables up to 40-year battery life.
Lower lifetime cost
Self-discharge is common to all batteries as every cell experiences some amount of chemical reactions, even when it is not in use or is disconnected.
Self-discharge can be effectively minimized by controlling the passivation effect, where a thin film of lithium chloride (LiCl) forms on the surface of the lithium anode to reduce the level of chemical reactions that cause self-discharge. When a continuous load is placed on the cell, it experiences a period of initial high resistance along with a temporary drop in voltage until de-passivation starts to occur, where the protective layer covering the anode begins to dissipate. The process of de-passivation repeats each time a continuous load is applied.
The level of the passivation is based on a number of variables, including:
• the cell’s current discharge capacity
• the length of storage
• storage temperature
• discharge temperature
• prior discharge conditions, as partially discharging a cell and then removing the load will lessen passivation effect over time.
High levels of passivation serve to reduce self-discharge but become problematic if it causes energy flow to become overly restricted.
Competing bobbin-type LiSOCl2 cells vary significantly in terms of their ability to harness the passivation effect. For example, the highest quality LiSOCl2 cells feature a self-discharge rate as low as 0.7% per year, able to retain nearly 70% of their original capacity after 40 years. Conversely, a lower quality LiSOCl2 cell can have an annual self-discharge rate as high as 3% per year. While this difference may not seem to be overly significant, over time it really adds up, as a 3% annual self-discharge rate causes 30% of the cell’s available capacity to be consumed every 10 years, making 40-year battery life unachievable.
High pulses drive wireless communications
Increasingly, IIoT-connected devices require high pulses of up to 15 A to power two-way wireless communications. Unfortunately, standard bobbin-type LiSOCl2 cells are not designed to generate high pulses due to their low-rate design. This challenge can be easily overcome with a hybrid solution that combines the use of a standard bobbin-type LiSOCl2 cell to deliver low-level base current with a patented hybrid layer capacitor (HLC) that generates pulses of up to 15 A. As cell capacity becomes largely depleted the HLC experiences a unique end-of-life voltage plateau that can be interpreted to deliver low-battery status alerts that reduce costs by enabling pre-scheduled battery maintenance.
Supercapacitors perform a similar role by generating pulses electromechanically rather than chemically. While commonly found in consumer electronics, supercapacitors are generally ill-suited for industrial applications due to serious limitations, including:
• short-duration power
• linear discharge qualities that do not allow for the use of all available energy
• low capacity
• low energy density
• very high self-discharge rates up to 60% per year.
Supercapacitors linked in series require the use of expensive cell-balancing circuits, which adds bulk and drains additional current to further reduce the operating life of the device.
Think long-term
With long-term deployments, the ideal power source should last for the entire life of the device so as to minimize the total cost of ownership by eliminating the need for costly battery change-outs. This requirement varies based on the application. For example, a device with a 10-year operating life may require a different battery chemistry than a device intended to last for more than 20 years.
With ultra-long-life deployments, choosing the highest quality cell can be essential to maximizing your long-term return on investment (ROI). Unfortunately, it can take years to clearly distinguish the difference between a higher-quality cell that features an annual self-discharge rate of under 1% per year versus a cell with a self-discharge rate of up to 3% per year. Be aware that the various test methods, theoretical models and algorithms used to calculate battery life expectancy tend to be highly unreliable over the short term by underestimating the passivation effect, as well as energy losses caused by prolonged exposure to extreme temperatures.
Sol Jacobs is vice president and general manager at Tadiran Batteries. Contact him at [email protected].
Latest from Power Supplies
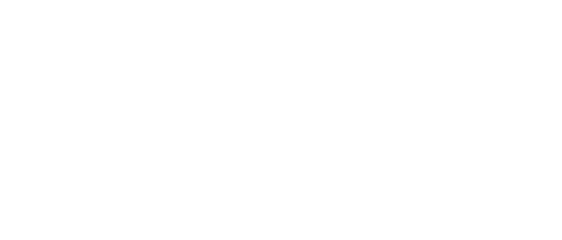
Leaders relevant to this article: